Historical Series: Photosynthesis
Unraveling Photosynthesis Step by Step:
Four Decades of Research in Theoretical and Computational Biophysics
A History in Four Parts
By Lisa Pollack
September 2012Part 4: From Molecule to Cellular Compartment
With the advent of the twenty-first century the team at Beckman was engaged in a whole host of photosynthesis work. Thorsten Ritz and Ana Damjanović were finishing up their explorations of light harvesting, and others in the group were expanding from purple bacteria to cyanobacteria and plants. In the middle of this first decade of the new millennium a chance meeting at dinner would bring Schulten closer to his dream of explaining the workings of a living cell from its constituent molecules. He would now go from molecule to organelle.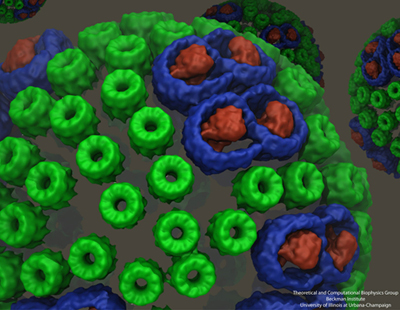
The chromatophore, a compact cellular compartment. LH1 proteins in blue surround the reaction center in red; LH2 are the green rings.
When Schulten moved to Illinois in 1988 he eventually met two scientists there at the university who also worked on photosynthesis: Antony Crofts and Colin Wraight, both from the School of Molecular and Cellular Biology. Schulten even published a photosynthesis paper in 1999 with Crofts. When Colin Wraight invited Schulten to a dinner in honor of a visitor from Sheffield, Schulten and the guest from England struck up a conversation about their respective work in photosynthesis. Neil Hunter, an experimentalist, was at that point in time imaging a structure called a chromatophore that was central to photosynthetic function in purple bacteria. Always on the lookout for collaborators, Schulten and Hunter decided to join forces to model the chromatophore.
Melih Sener, with a PhD in theoretical high-energy physics, started working on modeling the chromatophore in 2005. While high-energy physics and biophysics may seem like disparate fields, Sener had a clear objective for making the switch. “It's a little bit like the history of Don Quixote,” Sener explains. “He read knightly stories and attacked windmills. And I read the stories of all the heroes of nineteenth- and twentieth-century physics and when I realized that most windmills were already overtaken in high-energy physics, I decided that the most interesting problems are then in biological physics.”
A chromatophore is a complete cellular compartment that's part of a bacterial photosynthetic membrane. “It's essentially a machine that captures sunlight and converts it to chemically stored energy in the form of ATP, and it does so by a number of cooperating proteins,” Sener says. Similar to an organelle in eukaryotes, in bacteria this chromatophore is termed a pseudo-organelle. The chromatophore that Sener began working on is found in the purple bacterium Rhodobacter sphaeroides, contains several hundred proteins, and has a spherical shape that is in vesicle format, although not all chromatophores are spherical. One of the main tasks that Sener faced in the modeling project he light-heartedly compares to the forensics of road kill.
“The way these things are imaged is that you take the vesicle, and then squeeze it flat on a mica surface. Then you can scan it with an atomic force microscope,” explains Sener. With projection algorithms borrowed from geography, Sener deployed computing tools on a flattened patch to reconstruct what it might have looked like when spherical. In fact, Sener utilized experimental data from many sources, not only atomic force microscopy, to reconstruct the chromatophore. He cites as a major challenge the accrual and utilization of decades of experimental data. He culled from X-ray crystallography, NMR, electron microscopy, spectroscopic data, and, of course, the atomic force microscopy images from Neil Hunter's lab. Creation of the model, as shown above, is still an ongoing project for Sener in Schulten's group. For example, missing components need to be filled in, but experimental collaborators are working on some of these issues. To the best of his knowledge, however, this is most likely the first attempt to combine so much data into one structural, organelle-like model.
But this generation of the three-dimensional blueprint of the chromatophore was not the only goal. Sener removed the cap of the forensic scientist and replaced it with the cap of the physicist. His objective: use the tools of quantum physics to model light harvesting for the entire chromatophore. This stage, as well, presented multi-layered challenges to Schulten and Sener. One of them was the separation of scales, time scales as well as length scales. They wanted to describe the many processes involved in converting absorbed energy to ATP, but each step involved different time scales. For example, the first processes of light absorption and excitation transfer are femtoseconds to picoseconds, while the later processes of chemical transformations climb toward microseconds and milliseconds. Length scales also varied from describing electronic states distributed over a single molecule to as large as over the entire vesicle, 50 to 70 nanometers. “So naturally,” summarizes Sener, “you can't use one formulation, one language, one computational framework” when working with disparate scales.
The overall goal of this second stage of the chromatophore project is to compute the rate of ATP production as a function of incoming light intensity. “This required us to look at the chromatophore as a complete machine, where multiple processes communicate with one another,” Sener remarks. “And that's probably going to keep us busy for some time.”
While Sener and his many experimental collaborators were keeping busy modeling the chromatophore, Schulten put other members of his team on the project of self-assembly of photosynthetic membranes. As shown in the graphic above, the chromatophore consists of light-harvesting proteins (LH2 in green, and LH1 in blue with the reaction center in red) among its many components. Schulten had the idea that these light-harvesting proteins, which have a functional role within the chromatophore, may also help to shape the structure itself as the proteins of the chromatophore aggregate to form membrane indentations.
With papers published in 2008 and 2009, molecular dynamics calculations on three different bacteria showed that aggregates of LH2 complexes do seem to induce curvature, which is consistent with the curved chromatophore membrane structures seen in certain kinds or purple bacteria.
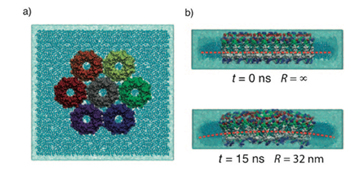
An example of the curvature induced in photosynthetic membranes, which is clearly visible after 15 ns of simulation. Image from Hsin et al., 2010.
Currently Danielle Chandler is continuing to study membrane curvature, with massive calculations that are pushing the limits of the simulation and visualization software produced in Schulten's group, namely NAMD and VMD. Her simulations encompass 20 million atoms, probably one of the largest molecular dynamics calculations in the world on a biological system to date. Membrane curvature is not the only physical property of study. “We're also interested in things like protein-lipid interactions, lipid mobility, etc.,” Chandler says.
The purple bacterium in question is Rhodospirillum photometricum, chosen because of the availability of atomic force microscopy data on the structure. But all this data presents major challenges for the researcher. One of the steps of this molecular dynamics simulation involves gathering coordinates for 20 million atoms, but the software VMD can only tackle a few million atoms. “John Stone, the developer of VMD in Schulten's group, had to invent a new file format to handle it,” continues Chandler. “The new format is a binary file formatso you can't open it in a text editor to look at it, but it can handle an arbitrarily large amount of data.” While the coordinates of Rsp. photometricum take up approximately 1.1 gigabytes, a trajectory file from a molecular dynamics run is about 4 terabytes large, and has to be stored on external hard drives. This calculation is already running on the Blue Waters supercomputer located at the University of Illinois at the National Center for Supercomputing Applications (NCSA). Completion of these massive computations will only bring the Schulten group closer to its goal of predicting the behavior of entire life forms from just their constituent atoms.
The Role of Quantum Coherence, Revisited
The final stage in this history of photosynthesis ends with a look at what the group at Beckman is doing most recently on quantum coherence in photosynthetic purple bacteria. In 1999 Schulten and his graduate students Ana Damjanović and Thorsten Ritz investigated how quantum coherence manifests in excitation transfer in the purple bacteria; at the close of this first decade of the new millennium Schulten returned to this topic, but in a much more comprehensive and elegant manner.
In 2009 graduate student Johan Strümpfer joined Schulten's group and faced a great challenge: find and implement a theory to explain excitation transfer in the pigments of purple bacteria that is free of all the assumptions that undergird the theory describing excitation transfer up to that point.
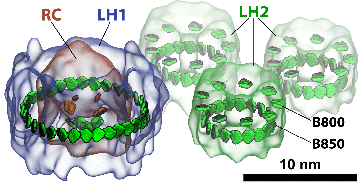
Light-harvesting proteins. Studies were done on how excitation is transferred from one ring to another, as well as within a single ring.
Coherence, which is a quantum phenomenon mentioned above and first described in the work of Xiche Hu, Thorsten Ritz and Ana Damjanović, means that two things, like molecules in a pigment ring, are correlated. “So coherence is like taking two things and making them almost one,” Strümpfer explains, “where one directly affects the other one instantaneously. There's no time delay, there's no exchange of anything, it's just an instantaneous thing.” For example, chlorophylls in a ring of a light-harvesting protein are involved in sharing excitation. “When chlorophylls share the excitation between them,” Schulten further clarifies, “it's not like the excitation hops from one chlorophyll to the next and then totally forgets where it comes from. Rather it remembers where it comes from.” This is one manifestation of coherence in photosynthesis.
One of the things Strümpfer had to account for was the thermal environment. Very often physicists like to do their calculations at absolute zero to reduce the complexity of a difficult problem. But Strümpfer did not have that luxury with living systems. “You have to work at room temperature, or the temperatures that these organisms experience,” Strümpfer explains. “And consider everything in place there: the membrane, the water, and the proteins surrounding everything. You know that those components are fluctuating with thermal noise basically.”
While taking into account all the molecules in a protein, and the lipid membrane it is attached to, and the water that surrounds it, may seem like a daunting task, Strümpfer and Schulten used clever mathematics to circumvent the problem of including those millions of atoms in the surrounding environment. The scientists again turned to Sir Anthony Leggett, who has studied quantum mechanics in a thermal environment. The elements of the surroundings, like water molecules or the lipids of a membrane, vibrate with a certain set of frequencies; Strümpfer used a distribution of frequencies that reflected the surrounding pieces and coupled the pigments he was studying to those frequencies. Strümpfer and Schulten basically took the ideas of Leggett and adapted them to their particular problem.
While Strümpfer had successfully unearthed a theory to explicate excitation transfer, implementation presented immediate challenges. Not only was the new theory hard to understand, writing code that ran fast enough was a hurdle. Strümpfer discovered that he had to educate himself very quickly about computer hardware and the nuances of writing efficient C++ code. This calculation required huge amounts of RAM, 200 gigabytes, and only one computer in the group met those conditions; but getting his code to take advantage of the huge memory was not straightforward. “I had to scramble and ask people all over the place how to do this. I needed to get it faster. I couldn't do the calculation unless I could get it done quickly.” Strümpfer eventually got his code working.
The graphic above shows a small segment of a photosynthetic apparatus, which includes a reaction center that is surrounded by an LH1 ring, and close by are three LH2 rings. The LH1 ring basically acts as a system of antennae, feeding excitation to the reaction center. But in the dark habitats that these purple bacteria live infor example, in the bottom of pondsmore feeder systems evolved, namely LH2s, to channel additional excitation energy to the LH1 and then to the reaction center. In the work Strümpfer and Schulten did on excitation transfer, they looked at transfer in two distinct forms: how excitation travels within a ring, and how excitation travels from one ring to another. And they found some surprising results.
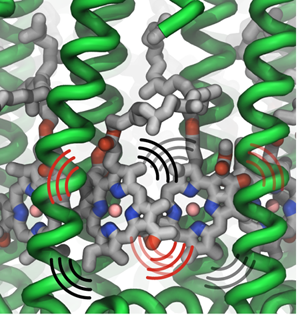
Thermal vibrations in LH2 proteins.
In considering how excitation moves within a ring, say within an LH1 ring or within one LH2 ring, Strümpfer looked at exciting just one pigment molecule individually in a ring and then observed how it settled. “Suppose excitation starts at one place,” spells out Strümpfer, “and then while this is still excited, another one also becomes excited. You have both of them excited at the same time, and then if it's very strongly coupled, then they're coherently excited. So then they act as one unit now, instead of being two separate things.” Strümpfer goes on to note that the description he and Schulten like for this inter-ring coherent behavior is to consider it as waves sloshing back and forth, or running around in the ring. “This is something that I struggled with immensely, actually,” admits Strümpfer, “because we don't have things that behave like this in our reality, if you can imagine.”
Strümpfer and Schulten found that within a ring complex, coherence plays an important role; but the hopping from one ring to another, for example from one LH2 to a different LH2, is much easier to visualize and compute. Excitation hops from one ring to another, and its mathematics is given by probabilities, but the excitation is never in both rings at the same time. “So that makes it easier, first, to imagine, and then second, to actually model physically,” remarks Strümpfer.
One of the major questions people have asked Schulten and Strümpfer about this work is how does quantum coherence then play a role in light harvesting. And Strümpfer calls the answer one of the most surprising findings to come out of their comprehensive study. “The effect is that having quantum coherence within one ring dramatically improves the rate at which excitation can jump from one ring to the next ring,” he summarizes.
Schulten is proud of his group's efforts on coherence, which started in the late 1990s and continues on today with the work of Johan Strümpfer. But this current work on coherence draws from some 30-plus years of Schulten and collaborators laboring to understand the story of how nature has fashioned photosynthesis to make it an efficient and elegant process. And Schulten's objective still remains to uncover the many steps of photosynthesis and clearly explain the findings to the research community. “There was a lot of work done, methodological developments and theory and so on, but the goal was to explain how nature effectively harvests sunlight,” summarizes Schulten on his last 35 years. “It's maybe still not complete, but I figured it out to a large degree.” And without a doubt, for what remains to be done, Schulten's group will be at the forefront in the years to come.
Part 1 | Part 2 | Part 3 | Part 4Comments?
For comments or inquiries, Lisa Pollack can be reached at the following: lpollack AT ks.uiuc.eduCitation
To cite this article, please use the following:Pollack, Lisa. "Unraveling Photosynthesis Step by Step: Four Decades of Research in Theoretical and Computational Biophysics." www.ks.uiuc.edu. 1 September 2012, from http://www.ks.uiuc.edu/History/photosynthesis/PhotoPart1.html
References
Stephen Jay Gould. Planet of the Bacteria. Washington Post Horizon, 119:344, 1996.
Martin Karplus. Spinach on the Ceiling: A Theoretical Chemist's Return to Biology. The Annual Review of Biophysics and Biomolecular Structure, 35:1-47, 2006.
Klaus Schulten and Martin Karplus. On the origin of a low-lying forbidden transition in polyenes and related molecules. Chemical Physics Letters, 14:305-309, 1972.
Klaus Schulten, H. Staerk, Albert Weller, Hans-Joachim Werner, and B. Nickel. Magnetic field dependence of the geminate recombination of radical ion pairs in polar solvents. Zeitschrift für Physikalische Chemie, NF101:371-390, 1976.
Hans-Joachim Werner, Zan Schulten, and Klaus Schulten. Theory of the magnetic field modulated geminate recombination of radical ion pairs in polar solvents: Application to the pyrene-N,N-dimethylaniline system. Journal of Chemical Physics, 67:646-663, 1977.
Zan Schulten and Klaus Schulten. The generation, diffusion, spin motion, and recombination of radical pairs in solution in the nanosecond time domain. Journal of Chemical Physics, 66:4616-4634, 1977.
Hans-Joachim Werner, Klaus Schulten, and Albert Weller. Electron transfer and spin exchange contributing to the magnetic field dependence of the primary photochemical reaction of bacterial photosynthesis. Biochimica et Biophysica Acta, 502:255-268, 1978.
Web of Stories. “Manfred Eigen, 108, The winter seminars.” Accessed April 12, 2012. http://www.webofstories.com/play/52329?o=MS
Web of Stories. “Manfred Eigen, 109, Why the winter seminars are unique.” Accessed April 12, 2012. http://www.webofstories.com/play/52330
Theodor Förster. Zwischenmolekulare Energiewanderung und Fluoreszenz. Ann. Phys. (Leipzig). 2:55-75, 1948.
J. R. Oppenheimer. Internal Conversion in Photosynthesis. Phys. Rev., 60:158, 1941.
William Arnold and J. R. Oppenheimer. Internal conversions in the photosynthetic mechanism of blue-green algae. J. Gen. Physiol., 33:423-435, 1950.
William A. Arnold. Personal Perspective: Experiments. Photosynthesis Research, 27:73-82, 1991.
Nobelprize.org. “Hartmut Michel - Autobiography.” Accessed April 13, 2012. http://www.nobelprize.org/nobel_prizes/chemistry/laureates/1988/michel-autobio.html
Nobelprize.org. “Johann Deisenhofer - Autobiography.” Accessed April 12, 2012, http://www.nobelprize.org/nobel_prizes/chemistry/ laureates/1988/deisenhofer-autobio.html
Nobelprize.org. “Video Player: Interview with Johann Deisenhofer,” Accessed April 12, 2012, http://www.nobelprize.org/mediaplayer/index.php?id=893&view=1
Nobelprize.org. “Video Player: Interview with Hartmut Michel,” Accessed April 13, 2012, http://www.nobelprize.org/mediaplayer/index.php?id=413&view=1
Johann Deisenhofer and Hartmut Michel. The photosynthetic reaction centre from the purple bacterium Rhodopseudomonas viridis (Nobel Lecture). Angew. Chem. Int. Engl., 28:829-847, 1989.
Johann Deisenhofer and Hartmut Michel. The photosynthetic reaction centre from the purple bacterium Rhodopseudomonas viridis. Science, 245:1463-1473, 1989.
Herbert Treutlein, Christoph Niedermeier, Klaus Schulten, J. Deisenhofer, H. Michel, Axel Brünger, and Martin Karplus. Molecular dynamics simulation of the primary processes in the Photosynthetic reaction center of Rhodopseudomonas viridis. In A. Pullman et al., editors, Transport Through Membranes: Carriers, Channels and Pumps, pp. 513-525. Kluwer Academic Publishers, Dordrecht, 1988.
Herbert Treutlein, Klaus Schulten, Christoph Niedermeier, J. Deisenhofer, H. Michel, and D. Devault. Electrostatic control of electron transfer in the photosynthetic reaction center of Rhodopseudomonas viridis. In J. Breton and A. Verméglio, editors, The Photosynthetic Bacterial Reaction Center: Structure and Dynamics, volume 149 of NATO Science Series A: Life Sciences, pp. 369-377. Plenum, New York, 1988.
Dong Xu and Klaus Schulten. Coupling of protein motion to electron transfer in a photosynthetic reaction center: Investigating the low temperature behaviour in the framework of the spin-boson model. Chemical Physics, 182:91-117, 1994.
Juergen Koepke, Xiche Hu, Cornelia Muenke, Klaus Schulten, and Hartmut Michel. The crystal structure of the light harvesting complex II (B800-850) from Rhodospirillum molischianum. Structure, 4:581-597, 1996.
Ana Damjanović, Thorsten Ritz, and Klaus Schulten. Energy transfer between carotenoids and bacteriochlorophylls in a light harvesting protein. Physical Review E, 59:3293-3311, 1999.
Thorsten Ritz, Ana Damjanović, Klaus Schulten, Jian-Ping Zhang, and Yasushi Koyama. Efficient light harvesting through carotenoids. Photosynthesis Research, 66:125-144, 2000.
Melih K. Sener, John D. Olsen, C. Neil Hunter, and Klaus Schulten. Atomic level structural and functional model of a bacterial photosynthetic membrane vesicle. Proceedings of the National Academy of Sciences, USA, 104:15723-15728, 2007.
Danielle Chandler, Jen Hsin, Christopher B. Harrison, James Gumbart, and Klaus Schulten. Intrinsic curvature properties of photosynthetic proteins in chromatophores. Biophysical Journal, 95:2822-2836, 2008.
Jen Hsin, Danielle E. Chandler, James Gumbart, Christopher B. Harrison, Melih Sener, Johan Strümpfer, and Klaus Schulten. Self-assembly of photosynthetic membranes. ChemPhysChem, 11:1154-1159, 2010.
Johan Strümpfer and Klaus Schulten. Light harvesting complex II B850 excitation dynamics. Journal of Chemical Physics, 131:225101, 2009.
Johan Strümpfer, Melih Sener, and Klaus Schulten. How quantum coherence assists photosynthetic light harvesting. Journal of Physical Chemistry Letters, 3:536-542, 2012.