Detecting DNA Orientation
with a Nanopore
test3
In a remote New Mexico dessert, Klaus Schulten met Amit Meller, who
told him a story of two DNA hairpins. The first one, when threaded
through the transmembrane pore of alpha-hemolysin blocks
the ionic current to 12 pA and escapes from the pore about three times
slower than the other one that blocks the current to only 9 pA. The
amazing fact about these experiments was that the two DNA hairpins
were identical in sequence. The only difference was in the global
orientation of the single stranded part of the hairpin in the pore of
alpha-hemolysin. Astonished by the outcome of their experiments, Amit
Meller and Jérôme Mathé sought an explanation from the modelers (Klaus
Schulten and Aleksei Aksimentiev). Cautious as the experimentalists
are, they did not tell the modelers which of the DNA orientations
produces the larger current blockade and which one escapes faster from
the pore. Below we describe our quest for the solution of the
puzzle. A formal report can be found here.
Deoxyribonucleic acid (DNA)
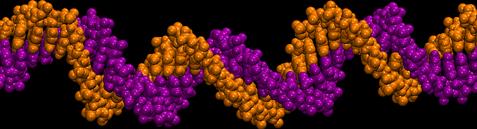
Double stranded DNA
Double stranded DNA (dsDNA).
In a living organism, a DNA molecule carriers genetic instructions for
synthesis of all the proteins required for building new cells or
sustaining the existing ones. DNA is usually drawn as a double helix,
in which two complementary but disjoint strands intertwine with one
another through a network of hydrogen bonds. DNA is a rather rigid
molecule: at physiological conditions, DNA curves at the length scale
of about 50 nm, which is 20 times the diameter of the
double helix.
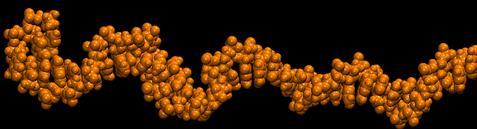
Single stranded DNA
Single stranded DNA (ssDNA).
For the sequence of DNA to be read, the double helix of DNA is split
open, exposing single DNA strands to DNA binding proteins. Once bound
to DNA, the proteins, in carrying out their functions, will crawl
along the DNA strand in one of two directions, toward DNA's 3' or 5'
end (see below). Single stranded DNA is much more flexible than double
stranded DNA and often folds onto itself forming a complicated
secondary structure.
DNA
direction. Yes, a DNA strand has a direction. It is determined by
the arrangement of the phosphate and deoxyribose sugar groups along
the DNA backbone. One of the DNA ends terminates with the O3-H group
(the 3' end), whereas the other one terminates with the O5'-H group
(the 5' end). Note that there are N-1 phosphate groups in an
N-nucleotide ssDNA. All sequences of DNA are usually written from 5' to
3' end. When forming a double helix, the complimentary DNA strands are
oriented in opposite directions.
The alignment of the bases can indicate the global orientation of a DNA strand
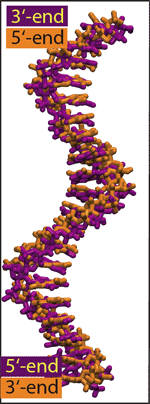
Alignment of two
poly(dA
20) strands that differ from each other only by
their global orientations.
DNA strands are
hard to destinguish in solution.
To reveal how the global
orientation of a DNA strand determines its escape time from a
nanopore, as well as the blockade of ionic current, we examined in
atomic detail conformations of DNA in solution and inside
alpha-hemolysin. Before running MD simulations, two identical
poly(dA
20) strands, different only by their global
orientation, were aligned with each other, as shown in the right
figure. The two strands appeared to have, overall, similar
conformations, the only difference visible being the ordering of the
backbone atoms: When moving along the strand from 5 to 3 end, a
phosphate group follows the deoxyribose sugar ring, the C5 carbon of
deoxyribose follows the phosphate. Reversing the order changes the
orientation of deoxyribose in the backbone. Although this change is
sufficient to disrupt the function of a dephosphorylative enzyme, when
put into the perspective of the alpha-hemolysin structure, it is an
unlikely reason for the observed directionality.
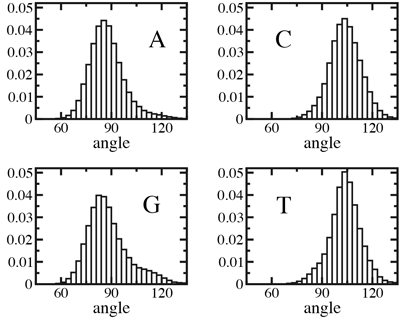
Normalized distributions of the angle between the base and the backbone of A, C, G, and T nucleotides in an unrestrained DNA strand. The average was taken over a 12.5-ns molecular dynamics trajectory.
First, the
experiments showed no evidence of a chemical reaction between
alpha-hemolysin and DNA; second, if one assumes that a DNA strand is
mobile inside the alpha-hemolysine pore (and our MD simulations
confirm this assumption), 12 sugars forming the backbone of a DNA
fragment confined within the alpha-hemolysin pore should be able to
sample the pore's interior regardless of the sugars' orientation.
Another possible explanation for the observed 3'- and 5'-
directionality might be that an ensemble of DNA conformations, by
itself, contains the information about the global orientation of a DNA
strand. To determine whether this is the case, we computed the average
angle between nucleotide bases and the backbone in a DNA strand
submerged in water. Our results shown at the left indicate that for
purine nucleotides (A and G) the most probable angle is ~88°, whereas
for pyrimidines (C and T) that angle is ~105°. From this analysis, we
conclude that local conformations can indeed indicate a global
orientation preference of a DNA strand, although the conformational
anisotropy in an unrestrained strand is small and depends on sequence.
Stretching DNA tilts its bases.
To investigate
the influence of the restricted pore geometry on the ensemble of
conformations adapted by a DNA strand, a poly(dA
11) strand,
submerged in a 1 M KCl solution, was confined by a mathematical
surface representing the shape of a cylindrical pore. Initially, this
pore was wide enough to accommodate the entire ssDNA strand the
backbone of which assumed a Watson-Crick helical form. During a 1.2-ns
simulation, the diameter of the pore was reduced from 3.0 to 1.0
nm. DNA atoms that lay outside of this surface were subject to a 10-pN
force directed toward the center of the pore. These forces adjusted
conformations of DNA to the shape of the pore with the DNA backbone
straightened; water and ions were not subject to the restraining force
in this simulation. In the left figure, we plot the resulting average
base-backbone angle as a function of the pore diameter. Similar
results were obtained with poly(dC
11) and
dC
7dAdC
3 strands. We noticed that when the pore
becomes 1.5 nm in diameter, the DNA bases of ssDNA start to tilt
collectively toward the 5' end of the strand. DNA bases were observed
to tilt toward the 5' end of the strand also when a 58-nt strand was
confined inside a phantom pore shaped like the alpha-hemolysin
channel. The same tilt was observed without confinement when the DNA
backbone was stretched (see the movies below).
Click here for a movie
(mpeg, 4.2M)
illustrating a molecular dynamics simulation of poly(dA11) strand in a shrinking pore.
Click here for a movie
(mpeg, 4.4M)
illustrating the stretching of a poly(dA11) strand by a
constant force applied to its 5' (bottom) end.
360,000-atom MD simulation reveals the mechanism of the direction-specific interaction between DNA and alpha-hemolysin
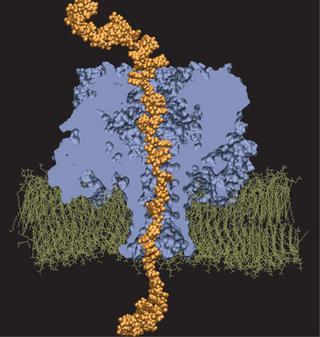
Molecular dynamics simulation of DNA permeation through alpha-hemolysin.
What consequence does the uniform tilting of the bases have
on DNA translocation kinetics and on current blockades? Intuitively,
one could equate driving ssDNA with an electric field
through the narrow constriction of alpha-hemolysin to bringing a tree
through a door: The tree moves more easily trunk first than tip
first (because branches tend to grow toward the tip of the tree).
Naturally, to determine the molecular mechanism underlying
DNA directionality, one has to visualize the translocation event
in atomic detail. Currently available computer resources only
allow MD simulations of a few tens of nanoseconds. However,
one can start a simulation with the DNA strand threaded halfway
through the pore and inspect over a few nanoseconds in how far
switching on an electrical field drives the strand for the next few
nanoseconds through the pore.
We have adopted this procedure to test the directionality
dependence of the field-induced transport of ssDNA. Two
systems, each comprising one alpha-hemolysin channel, a DPPC membrane,
a poly(dA58) strand, water, and ions, were subject to an
external electric field equivalent to a 1.2-V bias. In the first
system, the 5' end of the strand was located at the trans side of
the membrane; the system is shown in the figure. In the second
system, the 5' end was located at the cis side. The applied bias
forced DNA strands toward the trans compartment.
In the left figure we present how the DNA center of mass shifted
through the pore over the duration of 25 ns. One clearly observes
faster translocation for the DNA strand entering the pore 3 end first
from the cis side, in agreement with the experimental results
discussed earlier. Visual inspection of the DNA translocation events
with
VMD revealed the molecular mechanism
for the directionality of the translocation kinetics. In the case of a
field forcing ssDNA 3' end first through the pore, the bases remain
tilted in the same (3-to-5) direction; in the opposite orientation the
bases have to reorient to fit through the pore at its narrow parts.
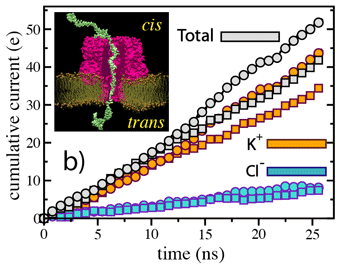
(cis)5-dA58-3(trans) data are
plotted as squares; (cis)3-dA58-5(trans) data are plotted as
circles.
We further computed the currents of K and Cl ions flowing through the
alpha-hemolysin during polynucleotide translocation according to the
procedure described
earlier. In
the right figurew we plot, in unitary charge units
(e =1.6·10
-19 C), the resulting cumulative currents. A linear
regression fit yields the average total currents of 330 pA for
(cis)3-dA58-5(trans) and 280 pA for (cis)5-dA58-3(trans), which are
24% and 20% of the open pore current, respectively. Although we
could not directly compare these values to measured currents, the ratio
of the currents, which is 1.2, is close to the experimental value
(1.3). We found that the total current is mostly generated by the flow
of K ions; the ratio of K to Cl current at a 1.2-V bias is 5:1. Inside
the alpha-hemolysin pore, the ions remain hydrated; their trajectories pass
through volumes free of DNA. The total charge carried by K and Cl ions
inside the alpha-hemolysin stem remained constant (15±1) in both simulations.
In conclusion, through
large-scale molecular dynamics simulations (and a lot of creative
thinking) we were able to reproduce the experiments results in silico and uncover the molecular
mechanism of the observed directionality. To our delight, simulations and experiments
converged!
Publications
Publications Database
Orientation discrimination of single stranded DNA inside the α-hemolysin membrane channel.
Jerome Mathé, Aleksei Aksimentiev, David R. Nelson, Klaus Schulten, and Amit Meller. Proceedings of the National Academy of Sciences, USA, 102:12377-12382, 2005.
Publications Database
Imaging alpha-hemolysin with molecular dynamics: Ionic conductance, osmotic permeability and the electrostatic potential map.
Aleksij Aksimentiev and Klaus Schulten. Biophysical Journal, 88:3745-3761, 2005.
Investigators
Simulations
Experiment
Related TCB Group Projects
Page created and maintained by Aleksei Aksimentiev.