Historical Series: Polyenes
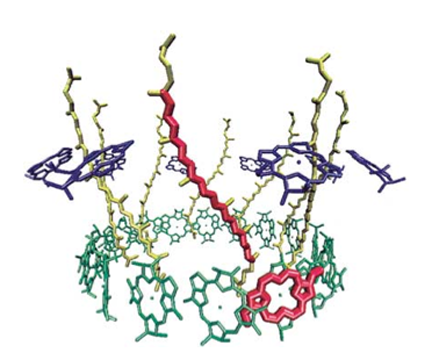
The upper red molecule is lycopene, a famous polyene. Its zig-zag shape is clearly visible. (Image is a light-harvesting complex.)
Uncovering a Hidden Family of States:
Quantum Biology of the Polyenes
By Lisa Pollack
February 2014See other related articles by L. Pollack
Nature is written in the language of mathematics.
Galileo
Luckily for Klaus Schulten, his dormitory was right across the street from his office at Harvard, because he had to wade through huge drifts of snow just so he could go to work. He had stocked up before the winter storm on spaghetti and canned sauce, much to his mother's horror. He was alone in Cambridge for the 1971-72 winter holiday, since he opted not to go back to his home in Germany for the break, but he had everything he needed. Including a riveting conundrum to solve.
With no one around the campus, not even his advisor, Martin Karplus, he was completely on his own and found himself awash in quantum chemistry theory. He was attempting to explain an anomaly two chemists there at Harvard had seen in one of their experiments. The two experimentalists were pretty sure the anomaly came from dirt not properly washed away from their apparatus, but even after cleaning and cleaning, the unexplained ripple in their data was still there. So they consulted the preeminent theoretical chemist on campus, Martin Karplus, for advice. And Karplus gave the puzzle to twenty-four-year-old graduate student Klaus Schulten. “I was just a new student of Karplus's,” recalls Schulten. “And so when you have a new student, you also have a new victim.” During the entire snow storm Schulten had in the back of his mind that he was attempting to explain the so-called “dirt effect.” With his intricate and lengthy derivations and computations he might just be calculating rubbish, for he could be verifying an experimental observation arising purely from dirt, which he had been clearly warned about. And that could result in a great humiliation for Schultenhe would have calculated something that didn't even exist.
But Schulten soldiered onward during that snowstorm, despite the caveat, carefully deriving his equations two or three times over to be sure he made no mistakes. His meticulous work was his inaugural venture into the world of original research, a world Schulten had wanted to join ever since he was a young boy. He wanted to become a scientist because he was hungry for discovery, and the snowstorm offered him the first opportunity in his whole life to uncover something new. “I was just really happy that I could do something for the first time, something on my own where people didn't know already the answer,” recalls Schulten about his work in the 1971 blizzard in Boston.
While Schulten was perfectly content to spend his vacation unraveling the mystery, it offered more than just a first shot at original researchhe was combining his two great loves: mathematics and nature. From a young age he felt compelled to study theoretical biology, for he loved nature but wanted to examine it with his imagination rather than in a formal laboratory. He'd always wanted to envision himself as a nano-sized ant and wander around inside a living cell. The project in the snowstorm definitely fulfilled his mathematical inclinations; but the molecules he worked on were also eminently important in biological systems. These molecules, the polyenes, are a versatile group of actors in chemistry and biology, and play a leading role in vision for all sighted species. Schulten had carefully orchestrated his choice of graduate school so that he could work with Martin Karplus on theoretical biology, and this project that Karplus suggested definitely had biological relevance. So Schulten's diligence to the problem at hand is no surprise, since he dreamed of doing original research in biology while relying on his mathematically oriented brain at the same time. It was literally a perfect storm for Schulten.
With his tireless efforts that winter break, Schulten would make a discovery that would only be the tip of the iceberg on polyene electronic excitations. And over the years he would go on to chart the actual extent of this new family of excitations that lurked beneath the surface. In fact, Schulten worked on this topic in three waves: as a graduate student in the early 1970s; and then immediately after that in a herculean feat from a physics angle; and by random chance late in his career he would revisit the polyenes again, this time in photosynthesis. This history touches on these three waves; it also explores in depth the path to the original discovery and its accompanying ridicule. In fact, in his second wave of research there was also derision from the scientific community. But through all the hardship, Schulten moved forward, for this research in quantum biology fascinated him on so many levels. Indeed, his early years as a youth and diploma student also offer hints about why Schulten rigorously pursued elaboration of the polyenes with such fire, and so it is there that we begin this narrative.
Books Get Teenage Schulten into Trouble
It was not long ago when the final authority on all topics rested solely in books. Before the paradigm shift of information switched from books to the internet, a young boy named Klaus Schulten was trying to educate himself on all the things he was most curious about, for school was not enlightening him sufficiently on these topics. Nature captivated him; mathematics captivated him; school bored him. He can pinpoint what instilled in him a love of natureit was his parents. They took him on hikes every weekend and every vacation. He was fascinated by the fish at the aquarium, and always had animals growing up. He wanted to know what made all these creatures living beings. While he wanted to understand nature more fully, he wanted to take a mathematical approach to study it, for he had a predilection and gift for all things mathematics. Today, this youthful passion of teenage Klaus Schulten might be called a fascination with theoretical biophysics. But back in the early 1960s he did not have that language, nor was anybody even really doing that kind of work. He just knew he wanted to combine his two great loves in his future career.
Books were a key source of information and inspiration, and sometimes even reprimand. When he was twelve or thirteen, Schulten discovered the preeminent Karl von Frisch through this biologist's 1960s book Du und das Leben (You and Life). “It showed wonderfully the wonders of life at many scales,” reflects Schulten about the book. “And, in particular, it stated that it all begins with the molecules in living cells, which at this point were still extremely mysterious.” Schulten particularly recalls Frisch pointing to molecules as the source of energy for living cells. Of course, these molecules are the familiar ATP (adenosine triphosphate). “I felt that was my calling,” notes Schulten. “I wanted to understand how life forms use energy to make ATP.” Moreover, Schulten explicitly remembers reading that the only way to understand molecules was to become a physical chemist. This information about molecules stayed with the young Schulten and would have dramatic implications for his path to becoming a scientist.
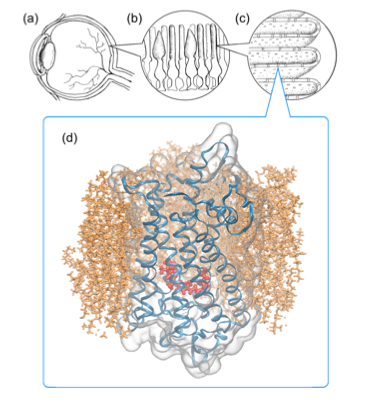
Rhodopsin in blue, found in the eye, a protein that intrigued young Klaus Schulten and that he would go on to study formally years later.
When Schulten was in high school (in Germany he went to what is called gymnasium, which is somewhat beyond the typical American high school experience), he was infamously known as “Professor.” No one ever called him Klaus. It seemed he always knew more than the teachers, which brought about resentment among some teachers and many comical moments for his classmates. It thus earned him that moniker, as well as no end to trouble. For example, in one class, students had to volunteer frequently to give reports about topics that were in the textbook. The topic one day was the eye and its accompanying lens behavior. The teacher asked for volunteers and only Schulten raised his hand. It was clear the teacher didn't want to call on Schulten, but knew all the other students were unprepared and would receive an F. So the teacher eventually gave in and picked Schulten but said, “Klaus, be reasonable.”
Schulten then explained every aspect of the lens behavior. When he was done with this aspect of the eye, he then said something like, “But it is quite interesting to considerWhat is the light actually doing in the eye? How is it received?” And he talked about the protein rhodopsin and how light is absorbed by it in the eye. Suddenly his teacher became furious, for what Schulten was discussing about rhodopsin was not in the textbook. The teacher gave Schulten a stern upbraiding. While Schulten's self-education was the only way to feed his widening curiosity, it certainly won him no favors with his teachers.
Schulten did not limit himself to the interesting books he could get in his small village, either. He went to the nearby university town and purchased their advanced science texts. He even gave physics lectures at home to his fellow high school students, on advanced topics. So when the time came for graduation from his gymnasium, and the annual essay competition for graduates sponsored by a local company, Schulten was ready to write a piece based on all the advanced texts he had been reading in physics. It was a very difficult competition, for Schulten recounts that often, in many years, there were not even any winners.
While Schulten won the competition that year, in 1966, decades later he would learn the truth about what really happened. His thirst for knowledge and the books he sought out to feed his curiosity left quite an impression on his teachershis physics teacher especially. Many years after graduation from his gymnasium, Schulten went back there to ask for a photocopy of the essay he wrote for the competition, for the school still had it in their files. Along with the essay, Schulten happened to come across a review of his essay from his physics teacher. This teacher claimed that the upper-level physics textbook Schulten based his essay on was so difficult, even for the advanced university students who were required to use it, that there was no way Schulten could have understood the text and based his essay on it. Clearly the committee that awarded the prize to Schulten found that his essay made good sense, and they were not swayed by the negative review from the physics teacher. However, upon receipt of his high school diploma and the renowned prize, Schulten was asked what he was going to study at the university. He told everyone: mathematics, physics, chemistry, and biology. “This was the final proof for my science teachers that I was insane,” Schulten says.
Racing Through University Diploma
After high school, Schulten went to nearby University of Münster for his diploma, and accomplished an unusual feat. He finished in the record time of three years; most students take about six to seven years to complete their diploma. Schulten had decided that he had to work in the middle ground between biology and mathematics and so chose to study physics at Münster as the best path. This exposed him to a wide range of courses in physics, mathematics, and chemistry. In fact, Schulten was eager for all the material he could unearth during his diploma. “I loved high school. I had all my friends there, but intellectually I was WAITING to go to university,” remarks Schulten. “So this was for me like a paradise; I couldn't take enough courses.”
In fact, taking a huge load of courses per semester was one thing that contributed to Schulten's rapid pace through the diploma. Another factor was that he was full of energy. But his high school years leading up to Münster were also fruitful. Schulten acquired many university textbooks in mathematics and physics, as mentioned above, and taught himself all the material he could get his hands on. This enabled him to jump right into upper-level courses his first year. For example, he took an advanced electromagnetism course his first year that was rumored to be outlandishly difficult. Most students were third, fourth, or fifth year. In fact, Schulten calls the professor “totally crazy” such that his lectures made no sense. So Schulten decided to teach himself the material. And Schulten figured that the crazy professor would have to give reasonable problems on the final. After the semester was completed, Schulten didn't even bother to go pick up the slip of paper that proved he passed the class, for he was certain a first-year student such as himself had little chance of passing. But then he started hearing rumors on campus: only two students had passed electromagnetism. When Schulten inquired further about the rumor, he learned that one of the students who passed the class was the crazy professor's advisee, and the other was some guy named Schulten!
While Schulten's boundless energy during diploma certainly played a major role in his completion time of a record three years, he says the professors at Münster were supportive as well. A good percentage of the faculty realized that the seven-year span necessary for acquiring a diploma was too lengthy. So Schulten's professors never forbade him from taking so many classes at one time. However, they did suggest he slow down, as they thought he might be missing things by working so quickly. “But somehow I felt I was not missing anything,” explains Schulten. “I was just doing what I wanted to do.” Schulten, however, did have leisure time during diploma. He would walk with his friends in the university's famed garden and they would all deliberate over their futures. “I discussed at length with my friends what I wanted to do with my life, and that is exactly what I do today,” says Schulten. His next move after diploma would bring him one step closer to his dream of exploring living cells at the level of atoms and molecules, which is what he envisioned for his future, but it would cost him.
From Hero to Villain
Schulten was nearing completion of his diploma, and his record-setting pace earned him quite a reputation on campus. He claims, however, that he went from hero to lowlife in the eyes of his friends and the physics community when he announced what field he was going to pursue in graduate school. This was 1969 and the hot fields of elementary particle physics and nuclear physics promised fertile ground for discovery; but Schulten told everyone he was going to pursue molecular physics. Boring! Perhaps Schulten was unconsciously remembering Karl von Frisch's book from childhood that claimed living cells were based on molecules and hence molecules were the key to unlocking the mysteries of life. However, his diploma thesis was in the field of molecular physics, and his experience writing it also cemented his decision to pursue the study of molecules for his PhD. And this augured the central role computing would play in Schulten's professional life as a scientist, stories told in more detail in this history of NAMD and this history of VMD.
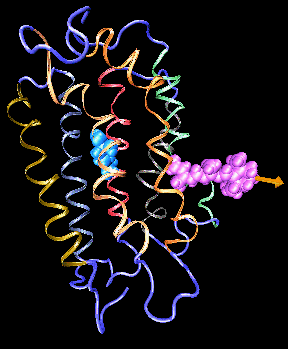
An example of a molecule, retinal (pink), which diploma student Klaus Schulten hoped to study in his future career.
The topic of Schulten's diploma thesis at Münster was the influence of the third atom in chemical bonding. When two atoms meet to form a bond and become a new molecule, in order for them to be caught permanently in an energy minimum, they need a third atom to take away some energy for the new molecule to form. After Schulten finished his thesis, he sat down with his advisor one day soon thereafter to discuss the results in the document. His advisor immediately honed in on the fact that Schulten left out an important case for the spin statistics under consideration. Schulten replied “No problem,” but the professor looked at Schulten as though something were indeed gravely wrong. However, it was lunchtime so the advisor asked if they could meet a few hours later to finish their discussion.
After their noontime break, Schulten presented a revised copy of the thesis to his advisor. “Where did these new results come from?” demanded Schulten's professor. The advisor thought Schulten would need months to derive the missing spin case and couldn't believe Schulten had calculated by hand the results over lunch. Schulten explained that it was truly no problem because he had written a general program for a computer and so it took just a few seconds to add a specific case. When Schulten explained about using the computer for his thesis, “the guy fell off his chair! He didn't realize what you could do with computers!”
Unbeknownst to his advisor, Schulten, completely on his own initiative, decided to use a computer to aid him in his diploma thesis work. But at this time in 1969, there were no desktop computers populating university science departments like there are today. In fact, it wouldn't be until the late 1970s and early 1980s that affordable desktop computers were even on sale for average consumers. Instead Schulten used a portable mechanical computer. At the university in Münster there were several physics institutes, their buildings spread across town. Schulten got an office to work in at the theoretical physics institute and couldn't believe his good luck. Not only was his office located atop a beer hall, which made all his friends jealous, but also inside it next to his desk lived an old mechanical computer on wheels. There is no telling what year it was constructed. However, Schulten taught himself to use it. “When I did my calculations it made this enormous noise,” recollects Schulten. “So I liked to use it usually after hours, when my other friends weren't bothered by the noise.” Schulten could literally hear it multiplying or adding. This mechanical computer with its audible parts indeed allowed Schulten to calculate for his advisor the missing case, which would have been a grave omission otherwise, over a mere lunch break. Not bad for a computer completely devoid of transistors!
The diploma thesis, which started Klaus Schulten on a long arc toward the world of computing, also helped him make a final decision about his choice of graduate schools. With guidance from the physics department there in Münster, Schulten got a fellowship from the Volkswagen Foundation to go to any graduate school of his choice, although he recounts that most students with the fellowship went to the United States. Schulten was accepted at Harvard, Stanford, and M.I.T. But in his thesis pursuits he had come upon the work of Martin Karplus, who was a theoretical chemist working at Harvard in 1969. Schulten not only studied the work of Karplus, but he heard through the grapevine that Karplus was interested in biology even though Karplus had been conducting chemistry research for most of his scientific career. With the knowledge that Karplus was interested in pursuing some kind of theoretical biology in the future, Schulten made his decision to join the physics department at Harvard, for Karplus would be close by and accessible to Schulten.
A Mystery Unfolds
Indeed, when Klaus Schulten arrived in Cambridge, Massachusetts for graduate school, Martin Karplus was just at that moment hatching a plan to retool his research from chemistry to something biology related. To this end, Karplus spent six months in the fall of 1969 in Israel, at the Weizmann Institute, to learn about what interesting topics in biology he could start studying in his own work. This turn to biology, his first love, is covered in detail in Karplus's autobiographical 2006 publication, “Spinach on the Ceiling: A Theoretical Chemist's Return to Biology.” Suffice it to say that when he returned to Harvard, Karplus was interested in vision, and especially retinal, the molecule in the eye that absorbs light.
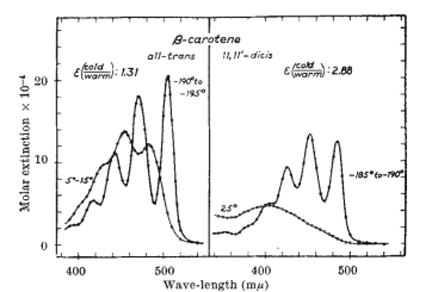
On the right hand side of the image, a typical "Boy Scout Salute" for the polyene called beta-carotene, as discovered by George Wald in 1959.
Retinal belongs to the family of molecules called the polyenes. These are long molecules that can absorb light, and have so-called pi electrons distributed over their length. They look like long zig-zag structures. While Karplus had just begun to study the polyene called retinal upon his return from Israel, he was soon confronted with a conundrum about another polyene in the fall of 1971. A fellow colleague in the chemistry department at Harvard, experimentalist Bryan Kohler, had been conducting spectroscopy studies on certain simple polyenes and noticed something suspicious. In his spectrum he saw a weak absorption that wasn't predicted to be there. Kohler's graduate student, Bruce Hudson, was conducting most of the studies. Both men, Kohler and Hudson, assumed that the small anomaly in the spectrum was just some dirt in the experimental apparatus that was not properly cleaned. So they cleaned again vigorously but the irregularity did not disappear.
What the experimentalists were seeing was an absorption spectrum with three expected peaks, and then in the low energy region, they observed the weak excitation they assumed was dirt. The three expected peaks, according to Schulten, came to be known as the “Boy Scout Salute,” for it resembled the three fingers boy scouts raise to each other in greeting. An example of a similar Boy Scout Salute in the polyene called beta-carotene, unveiled by George Wald in 1959, is shown in the figure. The reason the Boy Scout Salute was expected was because it was predicted to be there by molecular orbital theory. This quantum chemical theory assumes that all the electrons in a molecule are independent, and basically ignores the Coulomb repulsion between electrons. In fact, molecular orbital theory was known to work very well for the polyene electronic excitations. But no theory had ever clearly predicted the existence of a low-lying excitation in a polyene.
Kohler approach Karplus with the dilemma of this low-lying excitation in his polyene. Kohler had a suspicion it was something other than dirt. And, as Klaus Schulten was a new graduate student in the Karplus group, Schulten was given the task of puzzling out what was the cause of such a low-energy excitation in the polyene. However, Schulten says he was clearly warned that most likely the cause was just dirt not properly cleaned away. But Schulten, finally, had his first taste of the world of original scientific research and his first opportunity for discovery. He would soon be awash in heavy-duty quantum chemistry theory. And he was about to combine mathematics with biology, for the polyenes play a starring role in many aspects of life.
The Multifaceted PolyenesThey Are Everywhere
The polyene molecule is easily recognized as a chain of carbon atoms, which have alternating double and single bonds, and various groups of atoms attached at either end. But this simple explanation belies their complexity. Polyenes and their derivatives are found everywhere in living organisms: bacteria, archaea, plants, animals, and humans. In a 2013 article, Buckup and Motzkus call biopolyenes “the all-around Swiss army knife of nature.” One common subset of compounds with a polyene backbone is the carotenoids. A very famous carotenoid is beta-carotene, as it is a pigment that imparts vibrant color to carrots (which indeed influenced the moniker “carotenoid”). While beta-carotene is popularly known to be in foods like carrots and sweet potatoes, it is also found in parsley, broccoli, and onions, to name just a few. When humans ingest beta-carotene, the body oxidizes it and it becomes essentially two molecules of Vitamin A. The image shows beta-carotene and the two forms of Vitamin A that derive from the carotenoid. While humans make vitamin A1 from carotenoids, vitamin A2 is mostly confined to freshwater fish. The polyene backbone in the retinals is clearly visible, as the red indicates carbon atoms linked by alternating double and single bonds.
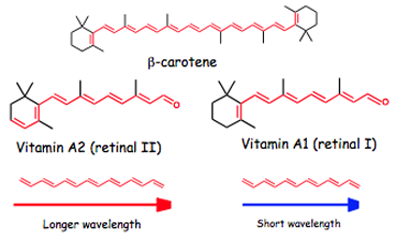
The polyene backbone of these molecules is evident in the red, which represents carbons connected by alternating double and single bonds.
The result of these double bonds means that polyenes are conjugatedthe upshot being that there are delocalized electrons in the molecule. These electrons (called pi-electrons) can be shared by more than one atom. In essence, conjugation is a primary reason that polyenes can make use of absorbed lighthence their importance in vision and photosynthesis. Klaus Schulten would in fact be consumed by the behavior of these pi-electrons when polyenes absorbed light.
Plants and bacteria can synthesize their own carotenoids from constituent parts. The human and animal body, however, cannot synthesize its own carotenoids, and hence has to consume them. An exception to this has recently been discovered in 2010 for aphids, where scientists postulate that a fungi gene for carotenoids transferred to aphids in the course of their evolution. Hence, aphids synthesize their needed carotenoids, but humans or birds do not. For example, the luminous color of flamingos comes from their extensive ingestion of blue-green algae, organisms replete with carotenoids. In captivity, flamingos have to be fed carotenoid supplements to reproduce their pink plumage. Humans, in fact, can turn orange by eating too many carotenoids, a condition (carotenemia) that is embarrassing but otherwise harmless, and is often seen in infants on the palms of their hands or their faces, usually from being fed too many strained carrots. There are over forty known carotenoids in fruits and vegetables. One subset of carotenoids (the carotenes) consists of alpha-carotene, beta-carotene, and lycopene, the latter receiving much recent attention as a possible anti-cancer agent, and commonly found in tomatoes. The other subset of the carotenoids (the xanthophylls) contains molecules important in the macula of the human eyelutein, zeaxanthin, and meso-zeaxanthin; the role of these carotenoids in age-related macular degeneration is currently under scrutiny. Lutein is found in abundance in spinach and kale, while corn is high in zeaxanthin.
Chemists first started intensely studying polyenes in the 1930sexamining their synthesis, their spectroscopic profile, and their theoretical descriptions. And the field of molecular orbital theory, in its infancy, used polyenes as test molecules to illustrate its defining principles and tout its successes. This is why the Boy Scout Salute was well understand in Kohler and Hudson's polyene spectrum, and why the low-lying anomaly was so mysterious. Interest in polyenes was renewed in the late 1960s as their role in vision became clearer. Chemists wanted to understand vision-related polyenes both spectrally and photochemically. Schulten got into the field during this second polyene renaissance, as he began his work in 1971. He, in fact, knew about the importance of polyenes in vision as he loved to hang out in the labs of George Wald and Ruth Hubbard, husband-wife biologists there at Harvard who were on the cutting edge of vision research.
The link to polyenes and vision goes back to the 1930s, when American George Wald conducted many experiments in Europe on the retinas of cattle, sheep, and pigs. Wald found vitamin A present in these retinas, which helped scientists explain the link that had already been noted between vitamin A deficiency and nutritional night blindness. In 1933 in Heidelberg Wald found that a novel carotenoid was present in the retinas of frogs, in a fortuitous discovery just weeks before he was scheduled to leave Germany due to Hitler's ascension to power. This novel carotenoid was similar to Vitamin A. It is now called retinal and has the familiar polyene backbone. Wald also found vitamin A2 in freshwater fish. For his pioneering studies in vision, Wald was awarded the 1967 Nobel Prize in physiology or medicine.
Retinal, a derivative of vitamin A, is part of the machinery of the eye and remarkable in many ways. The three types of eyesin the three different phyla of vertebrates, mollusks, and arthropodsmost likely evolved independently but all use the same form of retinal as their light absorber or chromophore. For example, in color vision, retinal is bound to a protein and that complex (iodopsin) is able to absorb light. Upon absorption, the retinal isomerizes and hence changes shape. This eventually induces conformation changes in the protein that retinal sits in and, after many intermediary steps, an electrical impulse to the brain is generated. In color vision in humans, there are three visual pigmentsred, green, and bluecorresponding to three different kinds of cone cells. Yet to sense these three regions of the spectrum, retinal remains the chromophore in each type of cone; what changes is the protein that retinal is connected to. Hence, retinal is well suited, with its polyene backbone, to spectral tuning, making color vision possible. The form of retinal used as the chromophore is not prone to spontaneous isomerization either, which makes it a stable pigment and assures that humans are not seeing false images in the dark. But when retinal isomerizes upon absorption of a photon, the structural change is significant enough to eventually generate a nerve impulse. Thus for many reasons, this polyene, retinal, is central to the sense of vision.
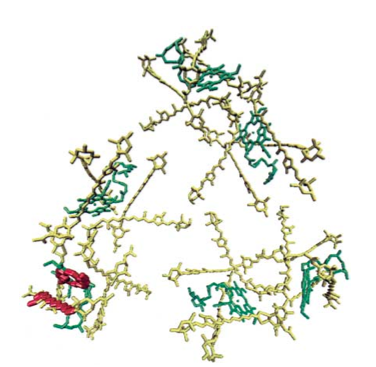
Carotenoids (yellow) and chlorophylls (green) both absorb light in the photosynthetic organisms called the dinoflagellates, whose protein PCP is shown.
As polyenes also play a critical role in pericyclic reactions and polymer science, they are indisputably keys to life (and especially vision) of humans and animals. Yet polyenes are not found only in living beings with eyesight. An extremeophile, Halobacterium salinarum (once known as Halobacterium halobium) thrives in water with a huge salt concentration, such as the Great Salt Lake and the Dead Sea, and can even live without oxygen. A form of retinal in this archaeon is key to its survival under anaerobic conditions. With a special protein and its accompanying retinal chromophore, this organism can use the sun's energy as a proton pump to create a useable form of energy for itself, namely ATP. This halophile manufactures its own retinals, unlike in animals, and employs them for more than just a proton pump. For example, a carotenoid in the archaeon's membrane (which actually is responsible for the pink color of the Dead Sea) can protect this life form from harmful effects of ultraviolet radiation. While carotenoids may be well known for the vibrant colors they impart, they are also important as anti-oxidants. Schulten relates that when he was in graduate school at Harvard in the early 1970s, he heard about exciting research just down the street at Tufts, which proposed a protective role for carotenoids. They were purported to be quenchers of excited oxygen, and this mechanism would indeed be confirmed and then elaborated on in the coming decades.
This brings in another important function of carotenoids in biologytheir role in photosynthesis. While most people are familiar with the chlorophylls in plants acting as the main antennae in the process of photosynthesis, carotenoids also play a role. They work as accessory pigments, absorbing that part of the sun's rays inaccessible to the chlorophylls, and transferring their energy on to the chlorophylls so that it can be used to make ATP. And plants are not the only biological systems that employ photosynthesis; bacteria also use it to garner energy from the sun. Little did Klaus Schulten realize, when he undertook that initial project on the baffling polyene behavior in 1971, that all his acquired knowledge about polyenes would some day benefit him in his future, when he would elaborate on a trick of nature. The mechanism of this trick is related to how energy is transferred from carotenoids to chlorophylls in purple bacteria photosynthesis, and we shall return to this topic in more detail. Suffice it to say, the mystery of the polyene behavior presented the young graduate student Schulten with a molecule so central to life for his explication, and it would become central to his life in just a short time.
Schulten Learns Science and Humility from George Wald
As his first dose of original research was centered on a polyene, Schulten was given a front-row seat to cutting-edge research centered on probably the most famous polyene aroundretinal. Namely, Karplus suggested Schulten go to the group meetings of George Wald and learn everything he could about vision, the new focus of Karplus's research. From this suggestion, Schulten got a precious view of the world of George Wald, a biologist there at Harvard. “He was a really nice fellow,” Schulten says of Wald. “ The group was very like family and he was always telling exciting stories. It was really fantastic for me to go to the group meetings.”
Schulten, however, almost lost the opportunity to attend the meetings, for he claims he was a typical “arrogant physicist” when he was young. During one of the Wald group meetings, a student was giving a talk, and presented a graph of wavelength versus another parameter, using for this visual a log-log plot. The student said something like, “Isn't this interestingit's on a straight line.” Schulten claims he said to this, after probably a whole slew of nerdy comments earlier in the presentation, “You found it very interesting? That's not interesting. Tell me which function doesn't fall on a line when you have a log-log plot!”
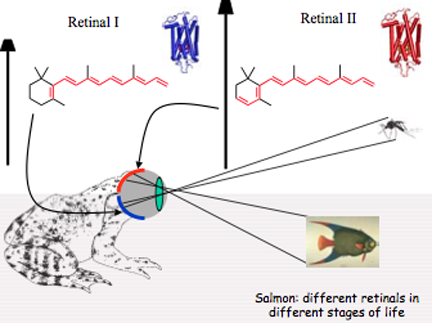
Klaus Schulten learned from George Wald about the two types of retinals in bullfrogs.
Schulten explains that he lived in an atmosphere, as a physics student, where mathematical insults were part of the culture of friendship. No one ever took it personally. But Wald was in the biology department, a distant cousin to the physics department, and the same rules didn't apply there. In fact, when Schulten made his retort about the log-log plot, Wald piped back with, “One more comment like this and I don't want to see you anymore.” Schulten immediately realized his remark sounded arrogant to all the biologists in the room, and that they didn't live in the mathematical world he inhabited. “So I found it really impressive,” Schulten says of Wald's stipulation, “that he told me if you are making obstinate, or insulting remarks, I don't want you here.” It was a good lesson for budding physicist Klaus Schulten, who wanted to work in the world of biology.
But Schulten learned more than this from Wald. He learned all about the polyenes retinal I and retinal II. One of the most fascinating things to Schulten was his lesson from Wald about bullfrog eyesight. A bullfrog sits in the water waiting for prey to appear, and has actually two visual fields. One field looks up at the sky, which is more blue, watching perhaps for a predatory bird, and the other field looks at the surface of the water, more red, perhaps for a fly to land there. So the bullfrog has adapted to using these two visual fields by employing two forms of retinal: retinal I and retinal II. For looking at the blue sky, the bullfrog uses retinal I. By adding two more carbons to create the longer retinal II, then this pigment can now absorb in the red and the bullfrog uses it for staring at water. Wald told Schulten that when bullfrogs are hibernating, they only have retinal I in their eyes, but come spring the eyes then utilize both forms. Wald further relayed to Schulten that salmon use both forms of retinal depending on if they are swimming in salty water or brackish water. “To me this was fascinating because I studied retinal,” summarizes Schulten. “And to realize, 'Oh there are two retinals!' And that one is absorbing a little bit more in the long, and that nature uses it actually. That was quite interesting.”
Consider the Most Complicated Equation
It was nearing the close of the 1971 fall semester at Harvard when Klaus Schulten was given the task to figure out what was causing the anomaly in the data Bryan Kohler was seeing in his spectra. Kohler was able to get very good results for the simple polyenes he was studying spectroscopically, by fitting the polyene in a crystal, which Schulten describes was like putting a polyene in between the pages of a book. With this method (a Schpolskii matrix), Kolher could constrain his polyene in a host crystal and thus could cool down his system considerably and get high-resolution spectra.
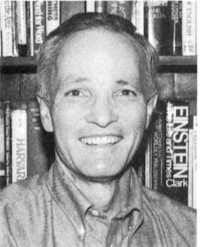
Bryan Kohler, a chemist who first identified a low-lying excitation in a polyene in his spectroscopy experiments in 1971.
Schulten had some ideas of where to begin because he and Martin Karplus discussed a few avenues of inquiry. As mentioned earlier, molecular orbital theory treats all the electrons in a system as though they are independent, and ignores the Coulomb repulsion between electrons. Schulten and Karplus thought that the Coulomb repulsion might be a factor to consider. Thus, Schulten was to explore the simplest case possible, that of two electrons interacting together, such that these two electrons are no longer independent entities but instead are getting excited together. Recall that the effect Schulten wanted to explain was a very low-energy transition, and when considering TWO excited electrons one would expect high energies. Double excitation probably meant double the energy. “So it was sort of little bit iffy, but that was the only thing we could think of at that moment,” Schulten explains about considering two electrons. “Perhaps there was an error in whatever was described and there was a chance to make a discovery.” Schulten then realized his job was to look at all the theoretical descriptions of polyenes and introduce the effect of the electrons doing something concertedly.
First Schulten decided, however, to derive all the results in the literature that already existed for polyene electron energy levels. His initial attempt was trying to re-derive the Boy Scout Salute, without considering Coulomb repulsion yet. “So I started out to do first the independent electron picture, and I didn't get it to converge,” he recalls. “I just took the simplest case, and I didn't get any reasonable results.” While Schulten was intent on studying molecules for the rest of his life, at this early time in his physics career he admits he didn't know one molecule from the other. He was desperate to figure out why his results didn't converge, so asked his office mates, graduate student Attila Szabo and postdoc Neil Ostlund (who together would later go on to write a classic text in quantum chemistry). They figured out for Schulten that he wasn't using the correct molecule in his program, for his polyene was one electron too short, not an even number of electrons, and so giving an unstable ground state. Hence the lack of convergence. When Schulten corrected the mistake, he found a beautiful result that agreed with all the literature.
But that was the independent electron picture. Now Schulten had to get down to the business of studying what two electrons are doing when they are no longer independent of each other. To do this, Schulten was going to use the theoretical technique called Configuration Interaction. When using this technique with two electrons, it is called “double excitation.” (For reference, when exciting one electron it is called “single excitation” and four electrons would be “quadruple excitation,” etc.) Accordingly Schulten, after he first got the independent electron picture out of the way and reached perfect agreement with the Boy Scout Salute, was finally ready to tackle the problem of two correlated electrons.
By this time in late December 1971, Boston was swamped in a snowstorm. There was almost no one around, and the snow made everything quiet so Schulten could focus well on his task at hand. In fact, he worked day and night, only stopping when his hand got cramped from holding the pen, as he was deriving all sorts of quantum mechanical rules over and over. He could barely wade through the snow to buy food. But that was not his biggest concern, the storm. “I was always worried that Hudson and Kohler would come through the door any moment and tell me, 'Oh, sorry, we found a better cleaning agent and now the effect is gone,'” Schulten reiterates.
Just like he had done for the independent electron picture, Schulten set out to derive all the preexisting results in the literature for double excitations. And he found a ton of errors in everyone's papersthings like sign errors and possibly typographical errors from printing. “But I had so much time there, that I was deriving all these formulas, long formulas,” recalls. “I needed big computer output to write it all down. And I did everything twice, very neatly, which is not my usual way.”
In essence, all the results in the literature that Schulten was studying were very messy to deal with. And in the midst of trying to sort out all the mess, Schulten realized something: one case of double excitation was completely absent. No one had ever written about it. This case was called “triplet-triplet.” To understand this, first consider what happens when an electron in a molecule is excited to a higher energy level. Electrons have something called spin, which is an intrinsic property of the particles. If two electrons occupy an energy state, then it is required that the two electrons have opposite spins, as is given by the Pauli Exclusion Principle. In a molecule before excitation happens, where the highest occupied level is populated by two electrons, these two electrons necessarily have opposite spins, one spin-up and one spin-down, a spin configuration in line with the rules of quantum mechanics. If one of those electrons gets excited to a higher energy level, it can move up and still retain its spin direction once it's in the higher level. This is called a singlet, which is based on its lone spin angular momentum value. A singlet would encompass a spin down electron in a lower state and a spin up electron in the excited level. But there is another way to excite an electron. When one electron in the pair moves up to the excited level, it can flip its spin. This is called a triplet (which is again, language from quantum mechanics, or S=1 yielding three spin angular momentum values.) So a triplet would encompass a spin up electron in a lower state and a spin up electron in the excited level. What Klaus Schulten realized in the muddle of all the literature about configuration interaction double excitations was that no one had ever written about promoting two electrons and simultaneously flipping each of their spins on the way up. He never saw a result for a triplet-triplet excitation. “So now I had this odd thing, but I knew my physics books and I actually loved the rotation group symmetry of it, so I knew that already pretty well as a young student,” he notes.
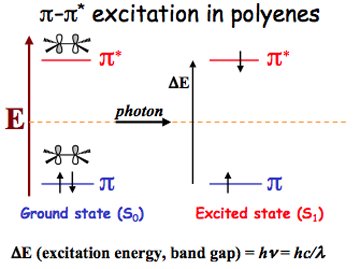
An example of a singlet excitation in a polyene, which has a spin up electron in the ground state, and a spin down electron in the excited state.
So Schulten decided to investigate this exotic case of triplet-triplet. He did the necessary math and it resulted in an extremely long derivation. “I thought that maybe others probably didn't do it because it is unimportant and the formula was so long that nobody wanted to calculate that correctly,” explains Schulten. Given all the errors in the literature, and the fact that the equation for triplet-triplet was extremely long, Schulten didn't want to make any mistakes. He was already deriving things two or three times to avoid errors, but then he realized he could take help from a mathematical rule of sorts. To move a single electron up, there were certain symmetries that result in the equations. And doing the same with double promotion, there were comparable symmetries he could check for. “I had this symmetry exactly, absolutely exactly, so I knew it was right,” he says. Schulten felt justified in moving forward with the calculation.
It was still during the snowstorm when Schulten finally got to the triplet-triplet calculation. And, lo and behold, he got a low excitation! Recall that the anomaly Kohler and Hudson were seeing in their data reflected a very low excitation. Schulten then had to figure out if his results were meaningful. He started to ponder excitations in general in polyenes. There were many published results in the literature for polyene singlet excitations; there were also published values for triplet excitations (here a single electron is moved up and flips its spin, not to be confused with moving two electrons to make triplet-triplet). Schulten realized that all the triplet excitations he saw were famously low compared to a singlet excitation for the same molecule. For example, in a polyene with eight carbons, a singlet state is 6.04 eV, but a triplet state is a mere 1.7 eV. Two triplets would be 3.4 eV, and this corresponded to the kinds of numbers that Schulten worked out for the polyenes he was consideringhis triplet-triplet example was still coming in much lower than a singlet. This gave Schulten confidence that he was on to something, and was not explaining dirt. “So I knew I did it correctly,” says Schulten about his laborious calculation. “And I knew why it worked.” As Schulten has coined it, he says he was “smarter afterwards,” for he could have pinpointed a low excitation just by looking at published individual triplet energies, but in research you often can only see the simpler answer in hindsight.
The Whole World Laughed at Them
By the time Martin Karplus returned from winter break at Harvard, Schulten had completed his calculations and explained that he indeed found a low-lying excitation. Karplus was very interested in the results and the pair couldn't wait to talk to Kohler and Hudson, whom they were still afraid might have found a better cleaning agent. But the experimentalists had not managed to eradicate the small excitation and were eager to publish that what they had seen in their spectra was backed up by theory and not just dirt. They wrote up their results quickly.
Schulten recounts that the paper was immediately rejected because the reviewer claimed the results were just the well-known dirt effect. The scientists were actually told the dirt was not in the polyene, but in the window of the cuvette, so it couldn't ever be cleaned away. But the Harvard scientists forged onward and eventually published their results. Two papers came out back-to-back in Chemical Physics Letters, one by the experimentalists Kohler and Hudson, and one by the theorists Schulten and Karplus, in June of 1972.
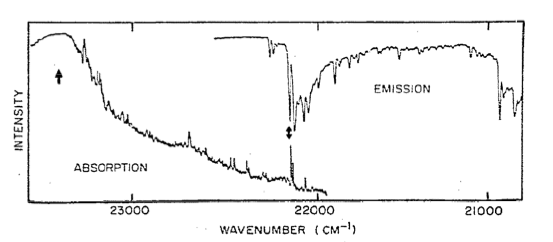
The infamous low-lying excitation Kohler and Hudson saw in their spectra, as indicated by the double-sided arrow.
The result of the papers, according to Schulten, was that the whole world laughed at them. Everyone claimed what the four Harvard scientists were explaining was the dirt effect. The critics were many, and Schulten claims the loudest laughter came from a famous physical chemist at the University of Chicago. Schulten still sees him today and they laugh about the dirt effect.
Schulten was undaunted. While the ridicule may have bothered some, as a young student Schulten knew he was on to something. He also claims that to have people laugh at him is better than saying something like, “interesting . . . but we knew it already.” The world expected the Harvard scientists to fall flat on their faces, but then a remarkable event occurred.
Although hard to believe, in 1972 lasers were a new tool in the worlds of chemistry and physics. Invented in 1960, they were actually touted as “a solution looking for a problem.” So use of lasers in chemistry for optical spectroscopy was rare in the early 1970s when Schulten was in graduate school at Harvard. But a laser was exactly the tool that helped to quell all the critics who claimed that Schulten and Karplus had derived a theory of dirt.
Part of the reason that Schulten's result about the low-lying excitation were controversial was because the transition to that energy level was forbidden. In fact, it was doubly forbidden. In quantum mechanics, there is a relatively straightforward way to tell if an electron can be promoted to a higher energy level. One uses the transition dipole moment. If the calculation with this operator gives a number that is zero, or close to zero, then this specific transition is deemed forbidden. Often you can tell if the quantity is zero just by looking at the symmetry properties of the integral. In the controversial low-lying state, there was actually a double selection rule, which meant that you have to obey TWO symmetry properties to make the transition allowed. As both of those properties were violated, the low-lying transition was doubly forbidden.
The truth is that a real molecule complicates this a bit. Symmetries are only maintained at very low temperatures when things stop moving, and for very ideal systems. And the experiments rarely can reach these ideal limits. So if each selection rule is 1% possible, then the resulting transition is 0.1% allowed. This is a weak transition, but not totally forbidden, for the molecule has not stopped moving completely.
But with a certain experimental techniquetwo-photon absorptionthe doubly forbidden transition becomes allowed, and the Boy Scout Salute then becomes forbidden. In this technique two photons are absorbed by the sample in question, but each photon is of half the energy, a key factor. With these low-energy photons, Kohler and Hudson, and many others, could then clearly see the low-lying transitions in the polyenes show up strongly in their spectra, and the Boy Scout Salute disappeared. Suddenly Schulten and Karplus had experimental evidence from two-photon spectroscopy to back up their theoretical results and were no longer a laughing stock in the science community.
In fact, as Schulten worked more on the polyenes, he started to uncover another interesting fact about them. The low-lying transition in the spectrum of Kohler and Hudson masked a pattern. As Schulten delved into longer and longer polyenes, he discovered that there was actually a family of low-lying transitionsthe low-lying excitation suddenly had brothers and sisters. Schulten discovered that the longer the polyene, the more low-lying excitations it had. A hidden family was unearthed, and it was definitely not the dirt-effect, as the family was clearly visible in two-photon spectroscopy.
The Joke about the Old Rabbi Training the Young Rabbi
Electrons are seemingly simple and ubiquitous particles, but trying to describe their behavior in a molecule has challenged chemists for years. With Klaus Schulten's 1972 work on excited electrons in the polyenes, he inadvertently waded into a fight that two camps of theoretical chemists had been having starting at least two decades before his discovery. The two camps were the molecular orbital people and the valence bond people. With Schulten's discovery of the low-lying electronic state, he realized that both theories had their merits when describing polyenes. No one camp could be declared the winner, a situation Schulten illustrates with one of his famous jokes:
An old rabbi is getting ready to retire, so starts to train a young rabbi. Sitting in the old rabbi's office one day, conducting their rabbi business, there was a knock at the door. A woman appeared and started complaining about her husband to the old rabbi. “He is always going to the pub. He doesn't help with the children. He is a really bad husband, etc.” The old rabbi looked at the woman and said, “You are right, you are right, you are so right.” The woman looked satisfied and left the office. Some time later another knock came at the door, and this time a man appeared. It was the husband of the woman. He started complaining bitterly about his wife. “She doesn't know how to cook. She doesn't keep house well. She complains bitterly to me, etc.” The old rabbi looked at the man and said, “You are right, you are right, you are so totally right.” The man looked happy and left the rabbi's office. At this point, the young rabbi turns to the old rabbi and says, “Ah, what is this? How can you say that both she is right and he is right? That is wrong!” And the old rabbi turns to him and says, “You're right.”
The point Schulten wants to make with the joke is that the excited electron in a polyene had aspects of both camps. In molecular orbital theory, Coulomb repulsion is rejected, but optically allowed excitations were still described well; for example, this theory solidly predicted the Boy Scout Salute. The valence bond theory took into account the repulsion of the electrons, and may have described the ground states of molecules very well, but totally failed to describe the Boy Scout Salute. This battle between the two camps was being waged in the days before powerful computers, and Schulten says that when he started studying polyenes in the early 1970s, it looked like the valence bond people lost the battle. “They were declared bankrupt,” says Schulten of the valence bond camp. “Whereas molecular orbital people, they were the big winners.”
But when Schulten started pondering the new low-lying excitations he had unearthed, he realized that the valence bond people had indeed described these excitations before, but just in unclear language and in such a way that people didn't recognize what it was. But Schulten recognized it. Hence, one family of excitations, the optically allowed ones in polyenes, was described well by molecular orbital theory. And the low-lying excitations that Schulten found could be elucidated with valence bond theory. “That is basically how the valence bond theory was revived through the work that I did,” Schulten explains. “Because it was theory non grata. But now suddenly they're both right.”
A Long-Standing Error Addressed
The debates over the two descriptions of electron behavior point to one thing that confronted graduate student Schulten back in the early 1970selectron behavior is not easy to characterize. As he was seeing, sometimes they behaved according to molecular orbital theory, and sometimes valence bond theory was preferred. “They can have various behaviors,” Schulten says of electrons. “And to describe the electrons in their full range of electronic excitations, this is actually a very challenging theoretical problem that EVEN TODAY is still being addressed to a large degree.” But Schulten wanted to make even more inroads on the new, once-hidden family of excitations that he had uncovered so diligently in the snowstorm. So he asked his good friend and fellow graduate student Iwao Ohmine to join him in a further elaboration on the polyenes.
As it turned out, Ohmine, in applied physics but also studying with Martin Karplus, had been working on polyacetylene. This molecule is a type of very long polyene, and in the early 1970s it was found to be conductive when doped, so it was a molecule that attracted much attention in the physics world. Ohmine was also looking at shorter polyenes in the course of his work. Ohmine and Schulten got to talking about their results for the polyenes called butadiene and hexatriene, and when comparing their results, realized that they had a publication there, their first one together. Thus began a fruitful collaboration between friends.
By 1973 Schulten had clearly realized that the low-lying electronic excitations were in fact a family, but as more experimental evidence came in about longer polyenes, Schulten's calculations were not matching what the experimentalists were seeing. Namely, as polyenes got longer, the excited states were growing smaller and smaller in energy. Yet calculations were showing just the opposite! Schulten and Ohmine decided to tackle this discrepancy between experiment and theory for their second publication.
They quickly figured out the reason that theory was showing high values for excitations as the polyenes became longer. In their method, configuration interaction, they were only able to calculate single and double excitations; computers were not powerful enough to include higher excitations like triple or quadruple. And this made the excited states unbalanced compared to the ground state; the ground state was well characterized, but the excited states were not. In essence, the ground state grew lower, and the excited states did not have enough electron correlation included to bring them down in energy, so the gap between ground and excited state was largemuch larger than experiment indicated. In fact, in Schulten's calculations, there was a divergence in the energy levels as the polyenes grew longer.
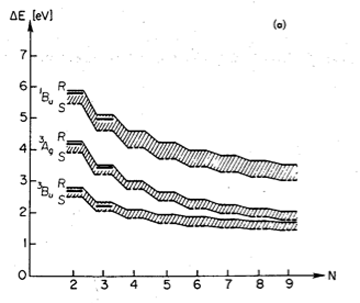
Using Renormalized CI, Klaus Schulten and Iwao Ohmine eradicated the divergence in energy as polyenes grew longer.
The two graduate students were at a standstill, since it was computationally unfeasible to include higher-order excitations. Then they had an idea: Use what is called a correlated ground state. Schulten likens it to considering the Coulomb interaction, but just for PAIRS of electrons; if there are three houses on a block, and inside each residence lives a couple, Schulten's approach considers the three couples separately, but not what happens if all the couples go on vacation together. In essence, instead of describing how one electron is affected by five others, this approach by Schulten and Ohmine considered just two electrons at a time, and utilized an operator for each set of pairs in the system. Schulten further simplified their procedure by making the operator take on an exponent form. He claims this is like factoring the complicated problem of many electrons into sets of pairs. And suddenly the calculations seemed realistic. But would Schulten's idea work?
The two, Schulten and Ohmine, immediately formulated the equations and wrote the appropriate computer code. But the computer was located in New York, at Columbia University. The students had a terminal in the Mallinckrodt Building at Harvard that was connected via a phone line to New York. “When jobs finished in Columbia, the printer in our terminal started printing the numbers on a roll of paper,” recollects Ohmine. “Almost all the time unwanted results came out, because of mistakes in the coding or printing.” When the printer returned garbage, Schulten and Ohmine would hurl epithets at it, probably in a mix of German, Japanese, and English, and then attempt to reprogram. Ohmine believes that he and Schulten stood by that printer for ten hours solid, forgoing sleep. Ohmine was drinking huge quantities of Coke just to stay awake. Finally, a beautiful result came through the printer, and the two men saw what they were hoping forno divergence in the excited energy levels. The culmination was a publication, in the Journal of Chemical Physics, later in 1978. The two decided to call their novel procedure “Renormalized Configuration Interaction.” Schulten claims it would be decades before most of the world appreciated what he and Ohmine did in that paper.
Ingenuity Applied to the Long Polyenes
Klaus Schulten was determined to pursue the study of molecules so he could gain a better understanding of biological systems. But when molecules become very large, most theoretical methods to describe their behavior are almost impossible to implement. However, Schulten, now in his first job after graduate school, wanted to delineate longer and longer polyenes and their related excitations. This was the late 1970s, when supercomputers for average scientists were not around to muscle through such demanding calculations for large molecules. Over the course of ten years, Schulten would use ingenuity instead of supercomputers to combat the problem of describing a large molecule, actually finding a way to simplify the near-infinite polyene description, but he would pay for it career-wise, as well.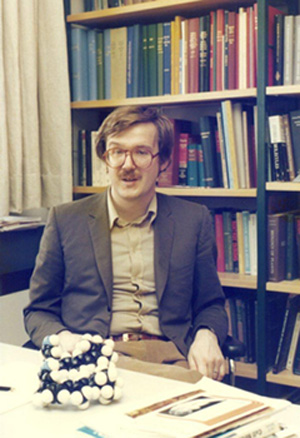
Klaus Schulten, in the mid-1970s, at his office in the Max Planck Institute for Biophysical Chemistry.
By 1974 Schulten was at the Max Planck Institute for Biophysical Chemistry in Germany, and very soon thereafter he was overseeing students. He would take on a young physics graduate student, Paul Tavan, and they would embark on an odyssey to systematically describe the new family of once-hidden excitations in the polyenes; this actually encompasses the largest effort in Schulten's three waves of polyene elucidation. The duo would go on to write over fifteen papers together on polyenes, and elaborate on their many aspects with very complicated and rigorous mathematics. “The real key was that we were so efficient in our description, that we could go to pretty long polyenes,” summarizes Schulten. This joint effort represented Schulten aspiring to go above and beyond the treatment of the low-lying excitation he had unraveled during the Boston snowstorman undertaking he says was easy compared to what he and Tavan accomplished.
When Schulten was at Harvard, for his dissertation he was able to describe the excitations in the polyenes butadiene, hexatriene, and octatetraene, which are four, six, and eight carbons long, respectively. Schulten says this was the absolute maximum he could do for his thesis, the eight-carbon octatetraene. He ran up against a wall; he realized as polyenes got longer there were more excitations to enumerate, but the math became harder and harder. Not only that, but the current theoretical descriptions were less accurate and introduced too many errors in the longer polyenes. “And the errors were interfering too much with the conclusions there in the simple description that we had,” Schulten contends.
The main focus for Schulten and Tavan would be to calculate the length dependence of the excitations. As already mentioned, when polyenes grow longer, more low-lying excitations arise. Short polyenes have a few low-lying excitations, while long polyenes have many. Schulten and Tavan wanted to know how all these excitations, lying so close together, were a function of the length of the molecule. The Harvard description Schulten worked out in the snowstorm was not giving the proper length dependence. Schulten and Tavan needed to go beyond the theory used at Harvard when Schulten was in graduate school to capture the proper behavior. At Harvard, what Schulten got was qualitatively illustrative, but quantitatively pretty bad. However, Schulten and Tavan took a near infinite system, seemingly impossible to reconcile, and turned it into a simple description to elucidate the length dependence. They improved the results both qualitatively and quantitatively for all polyenes, both short and long. But how did they capture the correct behavior of such large molecules?
As mentioned previously about the work done at Harvard by Schulten and his good friend Iwao Ohmine, to improve the accuracy of configuration interaction calculations, one needs to include not only singles and doubles, but triples and quadruples. While Schulten and Ohmine were unable to include the triples and quadruples, this was a task that Schulten and Tavan undertook in the late 1970s and 1980s. For example, in one publication in 1980, whose title begins, “An Efficient Approach to CI,” Schulten and Tavan confronted the ever-elusive problem of electron correlation. “You call it electron correlation because the electrons don't just move by themselves, but they move very much in coordination with each other, and in correlation with each other,” clarifies Schulten. In essence, electrons find ways of avoiding each other through coordination, an effect arising from the Coulomb repulsion. In order to make configuration interaction efficient, Tavan and Schulten used their extensive knowledge of group theory and symmetry properties to develop the complicated formulas they needed to derive. The sedulous efforts culminated in a tome of some thirty pages of intense mathematics. And the result was an acceptance letter from the Journal of Chemical Physics almost the next day! Schulten is quite sure the journal had no way of judging if the mathematics was correct, and instead chose to trust that Tavan and Schulten knew what they were doing with their complex formalism. While Schulten is pretty sure the journal did not read the thirty-page paper, he feels the community did not embrace it either. “We really made a major inroad in describing correlated electron excitations,” Schulten says of the 1980 article. “Everybody knew we could do it. It was published at great lengths, but it was almost ignored, this kind of heavy lifting mathematical work.”
But the team of Tavan and Schulten were not deterred. They were intent on getting the length dependence of the polyene excitations. And the element of their success, the trick to making description of very long polyenes simple again, hinged on a tool every undergraduate physics or math major learns at university: the Fourier transform. To help understand why it aided Schulten and Tavan, a music example best illustrates this concept. Suppose a pure tone is played of the musical note middle C, say on a computer or synthesizer. This musical note is described in physics as a wave that is a function of time. A musician with perfect pitch can tell you that what they are hearing is indeed middle C. The Fourier transform does the exact same thing that a musician with perfect pitch does! It reveals that the frequency of the given time-dependent wave is 261.63 Hz. If you Fourier transform an infinite sound wave, you get back one thingthe frequency. It is also true if you are transforming a violin string wave that is not infinite, but fairly long, that you get back the frequency. Tavan and Schulten applied the principle behind the Fourier transform to their very long polyenes. In normal space they had a large number of atoms to deal with, which were described by a length dependence variable. After the Fourier transform, they had a quantity that depended on what is called the wave vector, which is basically like the frequency of the excitation. The transform gave them the wave vector, and this wave vector they now used to describe the correlated excitations. Whereas a description of the system as a function of length was complicated and unwieldy, the description was greatly simplified as a function of wave vector. And they could now unveil the length dependence of the polyene family of excitations accurately, as a function of the wave vector. The result was a 1987 publication, and with 413 citations, one of Schulten's most highly cited in his career.
Outcasts in the Chemistry Community
Despite the tremendous efforts, rigorous mathematical treatments, and the models given for such long polyenes, Schulten says he and Tavan were considered outcasts at the time. The reason is their choice of methodology to explain the polyene excited states. “So everybody did ab initio quantum chemistry,” Schulten says of the 1970s and 1980s. “And only the low-lifes, like Klaus Schulten, did what was called semi-empirical quantum chemistry.” While ab initio treatment works on the premise that everything is built up from first principles, with a semi-empirical approach, approximations are introduced. Although the ab initio approach was considered the most accurate way to describe a molecule, chemists at the time could only apply it to very small molecular systems. Schulten's polyenes, as mentioned earlier, were very long in size. It would have literally been impossible to us ab initio methods on their polyenes.
While chemists decreed that ab initio methods were requisite, Schulten is a physicist, which gave him a different perspective. “So chemists basically saw the development of their field as going for accuracy,” Schulten reiterates. “Whereas the physicist were much more interested in qualitative understanding of electronic properties like superconductivity and electron behavior in solids. They didn't go this ab initio route.”
Schulten loves to point out that physicists still cannot calculate to high accuracy the conductivity of copper; instead they embrace a qualitative understanding. And despite this, physicists understand everything about copper wires without having a very accurate description. “Now it turned out in the end, we did the right thing because we wanted to understand biological compounds, larger compounds,” says Schulten about his polyene work with Tavan. “They were unreachable by ab initio methods. Maybe today they are, but definitely they were not for a long time.” But Schulten paid a price for it in his career. “So basically we did this work, which was very demanding intellectually,” Schulten notes. “But it was sort of being put in the drawer of the idiots.”
The Elusive Dark States
While the fruitful collaboration between Tavan and Schulten on polyene electronic excitations came to a close around 1987 with publication of their groundbreaking study, Schulten would unexpectedly revisit the polyenes about a dozen years later. This time, however, he was motivated to revisit the polyene family of hidden excitations at the request of an experimentalist who knew of the theoretical work of Tavan and Schulten. Schulten has collaborated with countless experimentalists in his career, but the majority of the time he has approached them. “So it was sort of a little unusual invitation,” he notes, “that the experimentalist comes to the theoretician and wants to write a joint paper.” But this experimentalist had verified a prediction made by Tavan and Schulten in their 1987 article, finding an excited state elusive to most experimental apparatuses for a decade. The lattice of coincidence that led Schulten to collaborate with the experimentalist Yasushi Koyama, and return to polyene excited states after a ten-year hiatus, is really rather remarkable.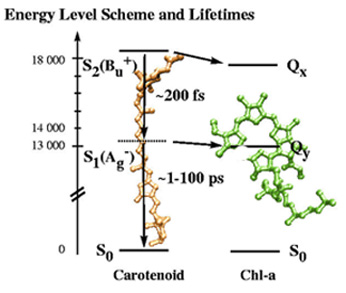
Tavan and Schulten predicted a whole host of energy states between S1 and S2.
One of the most important findings in Tavan and Schulten's work concerned their prediction of a whole class of excitations. The graphic shows the energy levels of carotenoids. What is important to take away from this graphic is that for polyenes, the levels called S0, S1 and S2 also hold. The state S2 is a strong absorber, and is easily identified in spectroscopy experiments. But Tavan and Schulten predicted a whole host of excitations between the gap S1 and S2, which they called “covalent excitations,” and which were elusive to experiment for quite some time, for they are undetectable by electron absorption or fluorescence spectroscopy. Somewhere along the way, these covalent excitations were labeled “dark states.” Schulten assumes they were coined “dark states” because they don't absorb light and they don't fluoresce.
However, in 1998 Japanese researchers, led by Yasushi Koyama, identified a dark state, that is, a state between S1 and S2, by carefully employing resonance Raman spectroscopy on spheroidene, a naturally occurring carotenoid. A few months before Koyama submitted his paper to Chemical Physics Letters on identification of the dark state in spheroidene, Koyama probably could not believe his luck to find that Klaus Schulten would be in Japan at a meeting in May of 1998. In an even greater piece of luck, Schulten already had a team of researchers assembled in Illinois who were actively working on photosynthesis, with part of their efforts devoted to carotenoids.
When Schulten finally met Koyama at the conference in Japan, Schulten agreed to take an excursion during the day to an earthquake museum, which Koyama drove them to. In the car ride the two men discussed their scientific interests, and Koyama asked if Schulten would like to collaborate. Schulten, while surprised, was thrilled at the invitation from Koyama. “He was really excited about this,” Schulten says of Koyama. “I was also of course excited but he was ten times more excited.” What the two scientists settled upon to write about was a trick of nature involving the chlorophylls and carotenoids that as yet had been sufficiently explained. And in this way, Schulten would revisit his work on the polyenes, for the properties of the “dark states” of Tavan and Schulten were key to unlocking the mystery of nature's trick.
Illuminating a Trick of Nature
Most people are aware of the central role that chlorophylls play in the process of photosynthesis. But in plants and in certain bacteria, they are not the only light-absorbers. Carotenoids, too, absorb light, acting as accessory antennae to the chlorophylls. Basically, carotenoids absorb light in that part of the spectrum outside the reach of the chlorophylls. Then carotenoids transfer that energy to the chlorophylls; it is the chlorophylls that funnel energy to the central powerhouse behind photosynthesis, the photosynthetic reaction center. In the photosynthetic reaction center, the energy captured by the chlorophylls and the accessory carotenoids starts its eventual conversion to a form of chemical energy the organism can then utilize to run its cellular processes. But carotenoids don't transfer their energy directly to the photosynthetic reaction center; they transfer energy only to chlorophylls. Then chlorophylls go on to transfer energy to the reaction center, energy either they absorbed or that they received from the carotenoids.
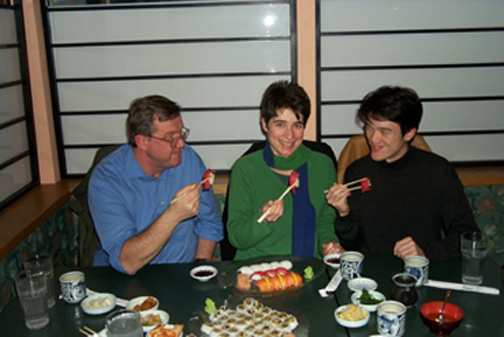
Klaus Schulten, with graduate students Ana Damjanović and Thorsten Ritz, who explained how carotenoids transfer energy to chlorophylls via symmetry breaking.
When Schulten was approached by Koyama for a collaboration, they decided to tackle a mystery seen in some photosynthetic organisms. At that time many people noticed that, relative to the amount of energy they absorbed, carotenoids in most species only were able to transfer about 50% of their absorbed energy to the chlorophylls. Looking again at the diagram of energy levels above, when the carotenoid absorbs a single photon, an electron jumps up to state S2. This state is so short lived that it can only transfer about 50% of its energy to the chlorophyll before the remaining 50% drops to S1, which is an optically forbidden state. The other half of the total energy then populates the S1 state until it is dissipated. This remaining energy goes nowhere. But then a strange thing happens in some species: Certain ones manage to transfer most of the remaining energy in S1 to the Qy state of the chlorophyll instead of wasting that energy. In the diagram, the transfer of energy to chlorophylls from carotenoids is shown by black arrows pointing from left to right. These arrows indicate that the carotenoids are coupled to the chlorophylls for energy transfer. In essence, most species only transfer about 50% of absorbed energy, from S2 to Qx, on to the chlorophylls; S2 is a strong absorber and a strong coupler. However, a few species additionally make the transfer from S1 to Qy. What could be the reason for this trick that only a few organisms learned? Koyama had been running experiments on this phenomena and worked on it for many years, but wanted a theorist's insight.
When Klaus Schulten was confronted with this dilemma, he called on his former knowledge of the dark states or covalent excitations delineated by Tavan and himself a decade earlier to help explain the enigma. And, in a further bit of luck, he had a pair of exceptional graduate students already fully engaged in the study of photosynthesis. Their pursuit of this topic, so central to biology and living systems, resulted in an effort that Schulten calls one of the best ever to come out of his group. So when Koyama asked Schulten to collaborate, Schulten already had a team assembled that was fully immersed in the field of photosynthesis and could effortlessly apply their skills to demystifying the trick of nature found in some carotenoids. A full catalog of Schulten's efforts in the field of photosynthesis can be found in this history of photosynthesis, including the remarkable achievements of his group around the turn of the millennium.
When Koyama approached Schulten during the earthquake museum excursion about a joint paper, the graduate students who were already immersed in a mammoth photosynthesis project at Illinois were Thorsten Ritz and Ana Damjanović. They then set out to tackle the mystery about why some species are able to transfer that remaining 50% of energy to the chlorophylls. Schulten's extensive knowledge of the forbidden states of the polyenes was one key to explaining the baffling coupling between the carotenoid and chlorophyll. Recall that the reason why the dark states are forbidden has to do with the symmetry of the polyenes or carotenoids. “It is a different kind of symmetry that comes from the dynamics of the electrons, basically how the electrons dance together,” clarifies Schulten. “This is literally true because it's called a pairing symmetry.” In most species that practice photosynthesis, the S1 state of the carotenoid is only weakly coupled to the Qy excitation of the chlorophyll, or in the language of quantum mechanics, the product of the transition dipole moments is zero or close to zero. When the carotenoid transition dipole moment is zero, there is no coupling between carotenoid and chlorophyll and no transfer of the remaining energy to the chlorophyll.
Working with Koyama, the theorists in UrbanaRitz, Damjanović, and Schultengave a clear explanation for why some species transfer the remaining 50% of the energy. The organisms that learned the trick were able to break the pairing symmetry, and suddenly the carotenoid transition dipole moment becomes a bit larger! But how? Recall that carotenoids are composed of a long zig-zag chain of carbon atoms, their polyene backbone. The symmetry breaking occurred when either a carbon in the chain was replaced by a heteroatom like oxygen or nitrogen, or by making a “hetero ring” at the end of the chain with nitrogen or oxygen in that ring. With the replacement by the heteroatoms, some species then were able to transfer almost 100% of their absorbed energy to the chlorophylls. And the key was an understanding of forbidden states that Schulten first elucidated in 1972. The result was a 2000 publication called “Efficient Light Harvesting through Carotenoids.” So, much to Schulten's surprise and delight, his thorough analysis of the forbidden polyene states came to benefit him years down the road, in demystifying a trick of nature.
Conclusion
This history began in a snowstorm, with a young graduate student given his first chance for discovery. But not only did Klaus Schulten have the wonderful good fortune to hit on a new finding in that blizzard in Boston in 1971, he also continued to elaborate on that finding in great detail for some twenty years. In these two decades of work Schulten undertook an exhaustive and intricate treatment of the low-lying polyene excited states with collaborator Paul Tavan, who has actually recently published again on the topic. In his 2012 article, “Electronic Excitations in Long Polyenes Revisited,” Tavan has recalculated polyene excitation energies using the so-called OM2 model. However, the paper also offers a general overview of the early work by Tavan and Schulten and briefly discusses the experiments verifying the states Tavan and Schulten predicted in 1987.
While polyenes were central to Schulten's life for so many years, the field of photosynthesis is a topic dear to Schulten's heart as well. And so he was able to combine these two subjects at the dawn of the new millennium, and revisit, one last time and quite unexpectedly, the polyene family of excitations. In fact, the intricate work on the polyenes fit right into the plan Schulten had for his life, to merge his love of biology with his love of mathematics. “So I had real fun with the highfalutin physics principles,” Schulten says about his long career with the polyenes. “And on the other side I worked on something that was eminently relevant for biology. So that combination was very appealing.” It is a combination that Klaus Schulten always dreamed of, and indeed, he has made it central to his whole life. This "polyene history" has shown repeatedly from the beginning to the end the opportunities for discovery through joint experimental and theoretical work. Schulten is happy to see that such opportunities that have advanced tremendously the fields of physics and chemistry now also bear fruitful discoveries in biology.
Other Related Articles
If you enjoyed this article, you might be interested in these other histories:History of Magnetoreception
History of Membrane Proteins
History of Bionanotechnology
History of Photosynthesis
History of NAMD
History of VMD
History of MDFF (Molecular Dynamics Flexible Fitting)
Comments?
For comments or inquiries, Lisa Pollack can be reached at the following: lpollack AT ks.uiuc.eduCitation
To cite this article, please use the following:Pollack, Lisa. "Uncovering a Hidden Family of States: Quantum Biology of the Polyenes" www.ks.uiuc.edu. 20 February 2014, from http://www.ks.uiuc.edu/History/polyene/
References
Martin Karplus. Spinach on the Ceiling: A Theoretical Chemist's Return to Biology. The Annual Review of Biophysics and Biomolecular Structure, 35:1-47, 2006.
Tiago Buckup and Marcus Motzkus. Time-Resolved Spectroscopy of Vibrational Coherence in Biopolyenes. Annu. Rev. Phys. Chem., 65:39-57, 2014.
Klaus Schulten and Martin Karplus. On the origin of a low-lying forbidden transition in polyenes and related molecules. Chemical Physics Letters, 14:305-309, 1972.
Bruce S. Hudson and Bryan E. Kohler. A low-lying weak transition in the polyene α,ω-diphenyloctatetraene. Chemical Physics Letters, 14:299-304, 1972.
Paul Tavan and Klaus Schulten. An efficient approach to CI: General matrix element formulas for spin-coupled particle-hole excitations. Journal of Chemical Physics, 72:3547-3576, 1980.Paul Tavan and Klaus Schulten. Electronic excitations in finite and infinite polyenes. Physical Review B, 36:4337-4358, 1987.
Tokutake Sashima, Hiroyoshi Nagae, Michitaka Kuki, Yasushi Koyama. A new singlet-excited state of all-trans-spheroidene as detected by resonance-Raman excitation profiles. Chemical Physics Letters, 299:187-194, 1999.
Thorsten Ritz, Ana Damjanović, Klaus Schulten, Jian-Ping Zhang, and Yasushi Koyama. Efficient light harvesting through carotenoids. Photosynthesis Research, 66:125-144, 2000.
Maximilian Schmidt and Paul Tavan. Electronic excitations in long polyenes revisited. Journal of Chemical Physics, 136:124309, 2012.